All published articles of this journal are available on ScienceDirect.
Finger Millet as a Sustainable Feedstock for Bioethanol Production
Abstract
The current trend in volatile oil prices, global warming and environmental pollution, has encouraged major consumers worldwide to sharply increase their use of “green” fuels. Bioethanol is usually obtained from the conversion of carbon-based feedstock. Bioethanol from biomass sources is the principal fuel used as a fossil fuels’ substitute for road transport vehicles. Bioethanol is predominantly produced by the sugar fermentation process, although it can also be generated by the chemical process of reacting ethylene with steam. Finger millet (Eleusine coracana) is also known as Ragi (India), Kodo (Nepal), Uburo (Rwanda), Kurakkan (Srilanka), Bulo (Uganda), Kambale (Zambia) and Tamba (Nigeria) and can be used as an efficient source for bioethanol production. Despite all its importance, however, finger millet is still grossly undervalued both scientifically and internationally.
This review observes current progress in bioethanol production from E. coracana feedstock and the effectiveness of various technological approaches for that.
The main aspects of ethanol production from finger millet seeds have been considered. Seeds, which are already used for brewing, are the most obvious variant of feedstock for ethanol production from this crop. The conversion of finger millet straw and agricultural waste into bioethanol has also been reviewed. Practical results of development and testing the tentative technology of sweet sorghum and finger millet combined processing into bioethanol are described. The concept of the tentative technology of bioethanol production from carbohydrate raw material of the first and second generations is suggested.
1. INTRODUCTION
Greenhouse gases (GHG) emissions and fossil fuel depletion are currently viewed as the major challenges of global industrial development [1, 2]. As it was predicted, the growing demand and consumption of fossil fuels lead to their gradual depletion within the following 40-50 years [3]. Apart from that, the burning of fossil fuels contributes significantly to overall GHG emissions [4]. In 2012, the National Oceanic and Atmospheric Administration (NOAA, USA) reported that annual average CO2 concentration in the air reached 392.82 ppm compared to 280 ppm of the pre-industrial era. The road transportation industry is the main contributor to air pollution, producing about 19% of the total global CO2 emissions (approximately 8 kg per 1 gallon of fuel) and 70% of total carbon monoxide emissions [5, 6].
Taking into account these facts, the EU Commission has planned to reduce GHG emissions by 40% by 2030 compared to the 1990’s level [7]. In 2019, the EU Commission took a more ambitious objective – to achieve climate neutrality by 2050 (Green Deal) [8]. Biofuels can become a highly important potential instrument for achieving this objective. Main discussions about the replacement of fossil fuels with biodiesel, bioethanol, biomethanol, biobuthanol, biogas, etc. started back in the 1980s [2].
Bioethanol is considered at the moment as one of the most promising substitutes for petroleum [1]. Basically, bioethanol is ethyl alcohol (C2H5OH) derived from renewable, mostly plant or algae, sources. It was also reported that the use of ethanol could provide only 66% of energy for burning in comparison with gasoline, but such fuel possesses a higher octane number [9] and generates lower GHG emissions [10]. In addition, ethanol is 15% more efficient for combustion than gasoline, since its molecule contains oxygen (as reported, about 34.7% in oxygenated bioethanol), which is absent in gasoline [11].
The idea of fuel vehicles with ethanol is not new. The earliest cases were reported by Nicolaus Otto in 1826 and 1876, who used alcohol for a four-cycle internal combustion engine [12]. After that, in 1908, Henry Ford’s Model T was firstly fueled with oil fuel-ethanol blend. Later on, ethanol-gasoline blends were widely used in the 1920s-30s and during WWII. In that period, such fuel (10% ethanol with 90% of gasoline) was called ‘gasohol’ [6, 12-14]. By the end of the 1990s, the first cars that could use 85% ethanol were designed and manufactured [12].
Nowadays, bioethanol was classified as an alternative fuel by the US Department of Energy in 2006, and its production in the USA, currently the top producer in the world, has been increasing every year and has doubled over the period from 2007 to 2013 [15]. The economic side of bioethanol production was discussed earlier, as well as the efficiency of different technological approaches [1], but the best solution has not been found yet. Nevertheless, ethanol has several advantages. For example, bioethanol contains a negligible amount of sulfur, compared to gasoline, which could reduce sulfur content and sulfur oxides emission if mineral fuel is mixed with ethanol [16]. Moreover, bioethanol could be used for raising the octane number instead of commonly used methyl tertiary butyl ether, which is harmful to human health [17, 18].
Bioethanol production is arbitrarily divided into three generations. The earliest, first-generation, is based on using different food crops as a feedstock. Starch from grains or carbohydrates from sugar crops are usually used in this case as a raw material for alcohol fermentation. The first generation bioethanol production is sufficiently developed, since it is based on the technology of alcoholic beverages production [1], competing with food industry needs. The second-generation bioethanol is based mostly on production from agricultural by-products, residues, trees, grasses and biomass leftovers, the feedstock that would not compete with the food production area. The use of polysaccharides (cellulose and hemicellulose) poses some difficulties in the production of alcohol as the necessary pre-treatment of such raw materials reduces the efficiency of the process. On the contrary, the biomass has a great potential for utilization because of its sustainable availability. It is anticipated that in the near future, the second-generation bioethanol might fully replace the first generation one [1, 3, 19]. The third-generation of bioethanol represents a novel approach, which is based on the use of algae and promises a higher bioethanol yield per hectare comparing with the efficiency of the second-generation feedstocks. However, there are various challenges to be overcome on each production step, for instance, lack of efficient, sustainable and cost-effective algae does not allow to implement the technology now [10, 12].
The second-generation bioethanol is very promising and feasible for implementation [1]. This approach requires several technological stages that have their own specifics. Since biomass and plant waste are mainly used as feedstock for second-generation bioethanol production, large amounts of sugars are not available for fermentation because these are polysaccharides - cellulose and hemicellulose. For this reason, before yeast fermentation, the biomass needs to be pretreated in biological, chemical, mechanical or physio-chemical ways [1, 14]. Depending on the plant source, the biomass should be processed using different methods, but generally, it is aimed at obtaining monosaccharides, suitable for fermentation and cleared from such unwanted components as lignin. At the stage of sugar conversion to ethyl alcohol, various yeast species or strains could be used. The choice of yeasts for fermentation is determined by several factors, like conversion efficiency (ability to convert the existing types of monosaccharides) as well as the biomass feedstock type [1, 20, 21]. After the final distillation step, a completely anhydrous end product – 99.5% EthOH, is received. This step is well studied, although, several different approaches are known to obtain the final product [1, 12, 22].
At present, one of the most important issues in the production of the second-generation bioethanol is a search for the most sustainable and high-yield plant sources. Major feedstock sources for the second-generation bioethanol are sugar cane bagasse, corn stover, various forest residues, sawdust, rice paddy, cassava, wheat, sugar beet, sweet sorghum, switch grass [12]. Cocksfoot grass (Dactylis glomerata), different cereal straw and peels/husk [10], Miscanthus and straw of other non-food species [2] could also be added to this list as potentially interesting sources [23]. As the search for new high-efficient feedstock is in progress, minor grains and cereals, also called orphan crops, which are grown locally in some regions or have been neglected, are now drawing a renewed interest [24].
One of such plant groups is represented by small millets that include finger millet (Eleusine coracana), foxtail millet (Setaria italica), kodo millet (Paspalum scrobiculatum), proso millet (Panicum miliaceum), barnyard millet (Echinochloa spp.) and little millet (Panicum sumatrense). These species possess several important benefits, including tolerance to drought and the ability to grow under low nutrient input conditions. In addition, their biodiversity is very high, which provides wide spectra of crop improvement approaches [25-27].
One of these small millets – E. coracana - has recently gained significant attention due to its valuable nutritional properties, ability to tolerate abiotic and biotic (pathogens resistance) stresses [28], including its potential to be used as a feedstock for bioethanol production [29]. It is also believed that the development of such undervalued but still perspective crops, such as finger millet, could lead up to the so-called “New Green Revolution” [25].
In this review, up to date progress in the development of bioethanol production from finger millet is considered. The effectiveness of various technological approaches for such production and future prospects for finger millet usage for these purposes are also reviewed.
2. FINGER MILLET: A GENERAL INTRODUCTION
2.1. Finger millet (E. coracana)
Eleusine is a small genus in the Poaceae family, which includes about 8 [30, 31] or 11 species, according to other sources, mostly of African distribution that includes one of the most interesting plants from the small millet group – finger millet [32, 33]. The etymology of the Eleusine genus name is tightly connected with the name of the Greek goddess of cereals, while the common, or species, name points on a finger-like branched panicle structure [34, 35]. Two finger millet subspecies, both tetraploid but morphologically differentiated, are identified: E. coracana ssp. coracana and ssp. africana [36]. Finger millet is shown in Figs. (1 and 2).
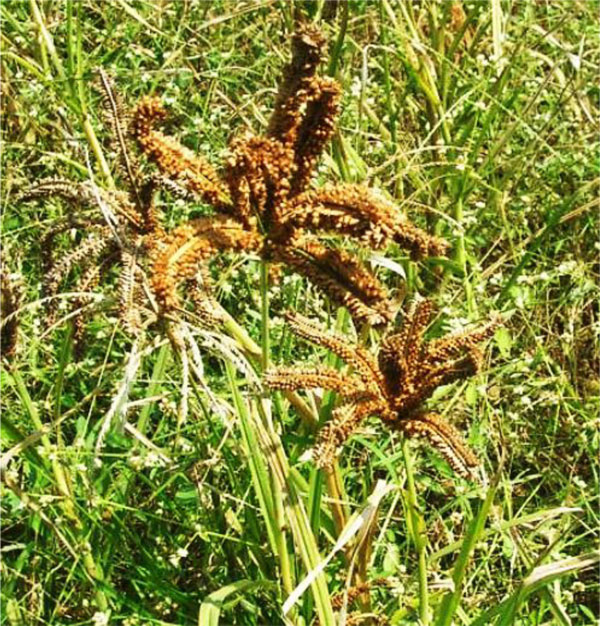
Finger millet is believed to have been domesticated approximately 5000 years ago in Africa, probably on the territory of Uganda, and later on, about 3000 years ago, it was introduced to India [37].
E. coracana, finger millet, or ragi, is known for its high nutritional value and a good taste that makes it an excellent source of carbohydrates like rice or wheat [38], rich in protein, fiber, minerals and vitamins [39]. This crop is also known to have perfect storage qualities: seeds can be stored unspoiled by insects or fungi for as long as 10 years [30]. This serves to provide an all-year-round food supply and grain reserves for years. For this reason, finger millet is also called a ‘famine crop’ [40].
Eleusine genus also includes some useful forage grasses (E. floccifolia, E. jaegeri, E. multiflora, etc.) and one invasive cosmopolitan weed – goosegrass (E. indica), which is considered to be one of the worst herbicide-resistant weeds with a notorious negative economic impact [31, 42-47].
Ragi is mostly cultivated in sub-Saharan Africa and Southern Asia regions, including India, China, Nepal, Kenya, Uganda, Sudan, Rwanda, Zimbabwe, etc [48], where it is an essential crop for indigenous farmers and poor people. About 4 mln. ha worldwide is devoted to finger millet cultivation [34, 49]. Provided optimal growing conditions, the seed yield of E. coracana can reach up to 5 t/ha, but usually, its productivity is much lower [36]. Finger millet is used both as animal fodder and for human consumption. E. coracana seeds can be ground into flour for preparing cakes, bread, or it could be used for soups, porridges. Moreover, finger millet malt is often produced for fermentation or brewing purposes [25, 30, 31, 33, 34, 50, 51].
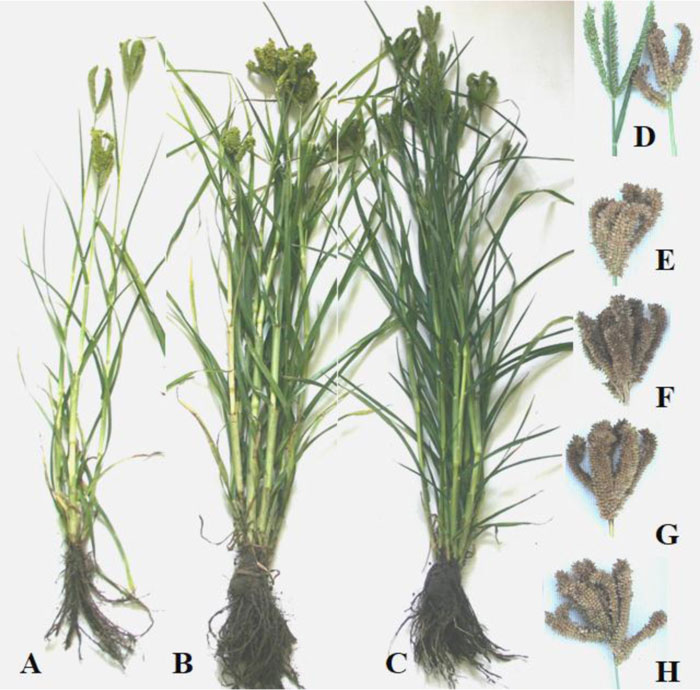
In addition, E. coracana is rather resistant to a wide range of abiotic stresses, especially to salt and drought stresses [25, 52, 53]. Furthermore, E. coracana is considered as “generally abiotic stress tolerant” plant [28]. One of the most valuable finger millet’s traits is an ability to grow under scarce nutritional resources available while keeping its grain nutritional contents high [35]. Therefore, the identification of novel sources of stress tolerance in this crop opens a wide range of opportunities for other agronomically important cultures. Thus, the ability of finger millet to grow on marginal lands with poor soil conditions could foster to reduce the competition between various purposes for its cultivation, e.g., for human food, industrial purposes or biofuel.
2.2. Breeding and Improvement of Finger Millet
Until recent years, efforts to breed finger millet have been very limited, despite the described benefits of this crop [31]. For this reason, E. coracana has retained a wide natural variety of genotypes [25], that can be used for breeding. A very good tool for that is to study finger millet biodiversity by inspecting various genebanks’ collections, which have been reported to have a huge number of its accessions [25, 48]. Looking at biotic stress resistance, several lines of finger millet resistant to blast disease have been identified in recent decades [28, 54]. This disease is caused by Magnaporthe grisea (anamorph Pyricularia grisea) and is the main factor limiting finger millet productivity.
The preserved biodiversity of E. coracana provides unique alleles for breeding [25], including a wide diversity of stress-resistance levels, which can be found across the genotypes [52, 52]. Marker-associated studies suggested that finger millet population polymorphism is very little [55-60]. It is unexpectable, taking into account E. coracana geographic distribution [25]. Furthermore, using restriction and amplified fragment length polymorphism (RFLP and AFLP), expressed-sequenced tags (EST) and SSR markers, genetic maps of tetraploid finger millet (E. coracana ssp. coracana) were generated [61] that could be applied in further breeding, aiming to improve various traits of this crop [31]. Therefore, molecular marker-assisted breeding is considered as a promising method for finger millet improvement [25].
Moreover, the whole genome sequence of finger millet available offers new ways for optimization of this process and accelerating new markers development [24]. Recently, genome sequencing of finger millet (ML-365 genotype) and transcriptome sequencing under induced low-moisture stress have been reported to identify potential drought-related genes, which is important for breeding high drought resistant genotypes [62]. In addition, high genome collinearity with foxtail millet and rice, even higher than with other Poaceae species, was shown for finger millet [62, 63]. This can provide new insights on further breeding directions and molecular markers development for the improvement of finger millet agronomic traits.
Besides that, strong progress is observed in the field of genetic engineering of finger millet, including both Agrobacterium-mediated and biolistic transformation [64-69]. It was also reported that protocols for effective in vitro finger millet regeneration are well developed at the moment [34, 70, 71]. Using the above mentioned techniques, E. coracana was improved for higher resistance to drought and salinity stresses [72-74] and disease [75]. These techniques were also used to obtain leaf blast disease resistant [76] and herbicide (dinitroaniline) resistant finger millet lines [77].
In general, most of the small millets usually suffer from low seed yields [69], which is less crucial for finger millet [36] but is a major challenge for breeding [25]. Additionally, concerning the bioethanol production, not only seeds, but also biomass is used for the production, which will be considered below. Green biomass productivity can be further increased by the improvement of the carbon fixation process [78], that can be achieved using the above mentioned molecular genetics techniques.
Apart from exploiting natural finger millet biodiversity, another promising method based on a somaclonal variability was proposed for the production of its new genotypes. This approach was described [79] where stable finger millet genotypes (Fig. 3) with normal tetraploid caryotype (2n=4x=36) were obtained. In that paper it was shown, that one obtained somaclonal genotype (SE-7) showed earlier maturation, higher seed and biomass productivity and a better germinability at lower temperatures [79], comparing with the referent finger millet variety – cv. Tropikanka [80]. Recently, this line (SE-7) was registered as a variety called ‘Yaroslav-8’ [81]. In addition, it was established that the SE-7 line possessed decreased levels of cytokinin-degrading enzymes, while cytokinin-synthesizing enzymes remained at the same level as in the wild type plant. This leads to generally higher cytokinin levels, stimulates meristem activity and induces increased finger millet growth [82]. Another promising somaclonal variant line of finger millet was obtained [83] and registered as a new variety [84] (Fig. 3).
As it was noted above, finger millet has very wide options of available biological tools that enable further improvement of various important agronomic traits of this crop: disease resistance, high abiotic stress tolerance, enhanced seed and biomass productivity, etc. Taking into consideration that finger millet is characterized by a very large, available (in genebanks) biodiversity, a number of well developed genetic engineering approaches and well-studied genome, E. coracana has a great potential to be cultivated now and be further improved for efficient and sustainable bioethanol production. Especially, considering such an important fact that finger millet could be cultivated on marginal lands with poor soil conditions, these characteristics may place finger millet out of the competition with other traditional crops, grown for human food.
3. BIOETHANOL PRODUCTION FROM FINGER MILLET
3.1. Ethanol Production from Finger Millet Grain
The most obvious feedstock for ethanol production from finger millet is grain, which is already used for brewing [25, 30, 31, 34].
Finger millet is known as a source of nutritionally valuable grain due to a high content of carbohydrates and protein [27, 85]. Proximate composition of finger millet grain, compiled
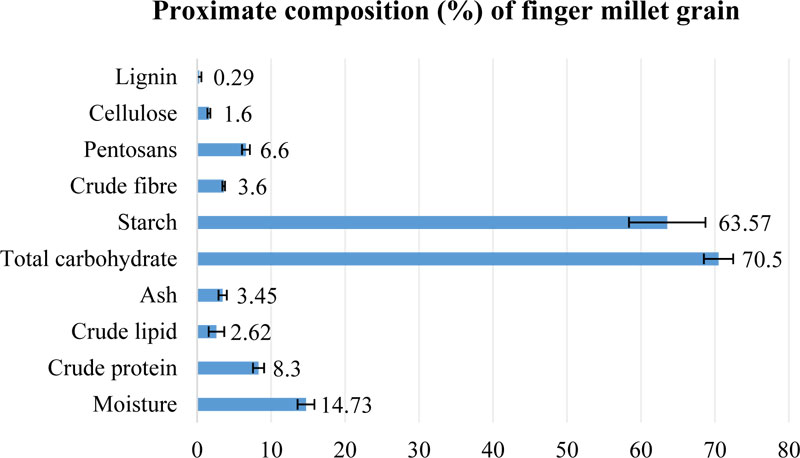
from different sources, is presented in Fig. (4) [27, 35, 50, 85]. The major component of finger millet grain is carbohydrate (68.6-72.6%), mainly represented by starch (60.01-69.5%). In general, carbohydrate content in finger millet grain is reported to be lower, compared with traditional crops like rice, barley or wheat; but, on the other hand, much higher than its content in grain of other small millets [27]. The content of other saccharides in finger millet seeds is very similar to the sweet sorghum composition. Additionally, finger millet grain possesses the lowest (1.5%) content of lipids in comparison to other millets or starchy crops [27].
Hemicellulose A and B of E. coracana are mainly composed of glucose, arabinose and xylose, while in seeds, the main sugars of the ethanol-soluble fraction are sucrose (up to 35%), fructose and glucose (both 15%), and raffinose (up to 12%) [85, 86]. As it was expected, the levels of free sugars are increasing during the malting process. Regarding the correlation of pentoses/hexoses in the water-soluble fraction of non-starchy polysaccharides, pentoses dominated in non-malted seeds, but after 96 h of finger millet seeds germination, the ratio changed to the predominance of hexoses [86]. It has recently been shown that the malt starch content decreased from 65% to 43% within 96 hours due to the action of amylolytic enzymes, in particular, α-amylase [87]. In this work, the pattern of starch granules degradation (larger granules were hydrolysed more efficiently than smaller ones) was also studied and it was shown that the malting process did not affect the relative viscosity of starch.
A traditional African brewing approach, which included the use of finger millet malt, was described previously [88]. Based on the conducted research, the author identified that finger millet possessed one of the highest α-amylase activity during malting in comparison with maize, wheat and pearl millet; only in sorghum seeds, this enzyme was found to be more active. It was also shown that the peak of E. coracana α-amylase activity was observed on the third day of malting [88]. This fact could explain the efficiency of the African traditional brewing approach, which includes adding of 3-days germinated finger millet seeds to the starch source (e.g., maize) treated with hot water to improve the output of oligosaccharides. In addition, it was reported that β-amylase is 2-3 times more active in finger millet germinated seeds than in sorghum [89]. The authors tested various mixtures of sorghum and finger millet malt (90/10, 80/20, 70/30 w/w) and, as a result, they observed a significant increase in free sugars levels and general fermentability in combined feedstock practically with unchanged viscosity and specific gravity values. Moreover, the output of ethanol from combined (70/30 w/w) mixed feedstock was up to 50% higher [89]. These facts provide strong evidence that finger millet malts could be used as a good additive to foster higher efficiency of alcohol obtaining from wide spectra of starchy feedstocks by simply creating malt mixtures. However, despite these positive outcomes, such an approach requires further elaboration before any final conclusions can be made.
Recently, the effect of different conditions for finger millet starch hydrolysis has been studied to create a feedstock suitable for bioethanol production [90]. For that purpose, powdered barley malt was added as a source of active α-, β-amylase in a proportion different to the ragi’s starch one. After that, the obtained mixtures were gelatinised and treated with high temperatures to activate α- (40-50°C) and β- (65-70°C) amylases. The authors of this paper observed increased levels of reducing sugars and identified the best conditions for hydrolysis: water dilution – 3 mL/g of finger millet starch mixed with 0.2-0.3 g of barley malt powder per 1 g of starch and hydrolysed for 3-3.5 hours [90].
Another approach to improve the ethanol fermentation
Technological Conditions | Obtained Amount of Ethanol | Yeast Species, Used for Fermentation | Refs. |
---|---|---|---|
30% w/v sugar; 4% w/v ragi flour | 14% v/v | S. cerevisiae | [95] |
30% w/v sugar; 4% w/v flour of malted ragi | 15% v/v | S. cerevisiae | [95] |
40% w/v sugar; 6% w/v ragi flour | 13.5% v/v | S. cerevisiae | [95] |
40% w/v sugar; 6% w/v flour of malted ragi | 15% v/v | S. cerevisiae | [95] |
Unsupplemented ragi mash (27.5% w/v of reducing sugar) | 9.1% v/v | S. bayanus | [96] |
Ragi mash (28% w/v of reducing sugar), yeast extract - 0.1% w/v, urea – 0.1% w/v, glycine – 0.1% w/v | 11% v/v | S. bayanus | [96] |
Ragi mash (27.8% w/v of reducing sugar), yeast extract – 0.3% w/v, (NH4)2SO4 – 0.3% w/v, MgSO4·7H2O – 0.1% w/v | 15.6% v/v | S. bayanus | [96] |
SSF: urea – 0.1% w/v, yeast extract - 0.1% w/v, magnesium sulphate – 0.05% w/v | 12.01% v/v | S. bayanus | [96] |
SSF: peptone 0.4% w/v, yeast extract – 0.6% w/v; glycine 40 mM | 13.1% v/v | S. bayanus | [96] |
process is to search for new microorganisms species capable to utilise sugars more efficiently. It was shown [91] that Zymomonas mobilis, which is usually used in palm wine production from wild dates, could produce significant amounts of ethanol and was able to convert finger millet hydrolysed flour even better than corn.
The technology that provides high ethanol yields in the final product of fermentation is very high gravity (VHG) sugar fermentation that is believed to be cheaper and sustainable in terms of energy requirements [92]. By definition, the VHG technology deals with the preparation and fermentation of mashes, containing 27 g of solids per 100 g of total mash weight [93]. It was reported that this technology makes it possible to obtain up to 16.2% v/v of ethanol in the final product when sake yeasts were used [94]. In the first study devoted to E. coracana application in VHG fermentation, an increase in ethanol concentration up to 15% w/v in the final product was reported if flour from malted finger millet was added to the media [95]. It was also found that fermentation time could be reduced from 5 to 3 days in that case. The authors tested different fermentation conditions (Table 1), including different temperatures, sugar concentrations and various types and amounts of finger millet flour. The highest ethanol output was obtained when the respective amount of flour of malted seed was added into the substrate, but taking into account the amount of feedstock – the most efficient medium was at 30% w/v sugar with 4% w/v malted ragi flour. In all cases, yeast growth stopped after 50 h of fermentation. The best optimal temperature to obtain these results was 25°C.
Recently, the finger millet based VHG fermentation technology has been further improved [96]. In that study, Saccharomyces bayanus was used for ethanol obtaining; α-amylase treated finger millet mash with 27-28% w/v of reducing sugars was taken as the medium. Fermentation was conducted at a temperature of 30°C. Additionally, the efficiency of three different conditions was tested for separate hydrolysis and fermentation reactions (Table 1) [96]. The first reaction type with non-supplemented mash showed the lowest productivity with 51.1% of fermentation efficiency and only 9.1% v/v of ethanol yield. Mash supplemented with glycine, urea and yeast extract produced higher, but still rather low alcohol output – 11% v/v, while mash with yeast extract and mineral additives allowed to obtain 15.6% v/v ethanol in the final product (fermentation efficiency – 86.6%). These results suggest that the VHG fermentation technology could solely be based on finger millet’s reducing sugars medium [96], contrary to a study [95], where E. coracana was only a source of flour as an additive to free-sugars based medium. The authors also tested simultaneous saccharification and fermentation (SSF), which was found to be less efficient, than separate hydrolysis and fermentation [96]. The SSF approach showed a lower efficiency and ethanol output (only 12.01-13.1% v/v) in the final product, even with the addition of yeast nutritional supplements in media (urea/yeast extract/magnesium sulphate or peptone/yeast extract/glycine). The fermentation conditions are presented in Table 1. Recently, these authors continued investigation of the influence of nutritional supplements on the efficiency of SSF VHG fermentation based on the finger millet mash [97]. Their mathematical model showed that the SSF process could give only up to 13.9% v/v of ethanol even under optimised amounts of supplementary components. The real ethanol outcome (13.1% v/v), obtained earlier by these authors [96] (Table 1), was very close to modelled one.
In a review of recent advances in HG and VHG ethanol fermentation, it was reported that it was possible to obtain up to 17.6 v/v % of ethanol in the final product [98], but that result was not achieved for finger millet yet. In addition, it was mentioned that the VHG fermentation approach could also be suitable for pre-treated finger millet biomass fermentation [98]. However, to our knowledge, up until now, no research on this topic has been reported.
In the above-reported investigations, the major efforts were dedicated to achieving the highest possible output of ethanol via the fermentation. Apart from that, separate research was aimed at obtaining more ethanol output per hectare of the cultivation land by increasing grain productivity of finger millet [83]. It was reported [83] that varieties bred from finger millet somaclonal variants (previously described [79, 82]) showed the higher output of total sugar per hectare (cv. Yevhenia – 900 kg/ha; cv. Yaroslav-8 – 880-1002 kg/ha) compared with original reference genotype (cv. Tropikanka – 500 kg/ha). Subsequently, further experiments on ethanol production were based on cv. Yaroslav-8 as a grain source [83].
Supplementation variant | Seed yield, t/ha | Bioethanol yield, L/ha |
---|---|---|
Control (no supplementation) | 6.20 | 2400 |
Phosphobacterin | 8.11 | 3120 |
Phosphate, nitrate and potassium mixture supplementation (35 kg/ha) | 9.85 | 3800 |
For ethanol obtaining, seeds were firstly ground to obtain the seed powder. Then mash was prepared by mixing water (L) with seed powder (kg) at a 7:2 v/w ratio. After that, the mash was incubated with thermostable α-amylase (Thermamyl SC, Novozymes) for 3 h at 80-85°C, and then saccharified with a commercial enzyme mixture with glucoamylase activity (SAN Super 240L, Novozymes) at 30-65°C, pH 4.0-6.0. The obtained mixture was then fermented with S. cerevisiae for 72 h. The final ethanol concentration received was 9.5% (v/v). Since the total ethanol yield per hectare was the main objective of this study, subsequent experiments on increasing finger millet seed yield were conducted by adding mineral substances to the soil [83]. Ultimately, all harvested seeds were converted into bioethanol and final alcohol yield was estimated (Table 2).
Cultivation of the above-mentioned finger millet variety without mineral supplementation provided only 6.2 t of seed per ha and 2400 L of ethanol output. In the alternative case, finger millet variety was cultivated on a phosphate-enriched soil. This was carried out by introducing a bacterial inoculant, called “phosphobacterin”, which consists of mass spores of Bacillus megaterium var. phosphaticum [99, 100]. The soil supplementation with phosphate-producing bacteria increased the seeds yield to 8.11 t/ha and raised the ethanol output to 3120 L. Cultivation of finger millet on fully supplemented soil (phosphate, nitrate and potassium mixture, 16:16:16) resulted in almost 50% increase of production (up to 3800 L/ha of ethanol) [83]. These results revealed an extra potential for elevating the bioethanol production efficiency from finger millet. Moreover, the implementation of other described above fermentation technologies can help to further increase ethanol production from this crop.
3.2. Conversion of Finger Millet Straw and Agricultural Waste into Bioethanol
A more advanced technological way to produce bioethanol is connected with the use of biomass as a feedstock. Utilization of straw and agricultural waste, such as husk, could help to avoid competition between food and non-food use of cereals. To our knowledge, rather limited information is available about technological approaches of E. coracana biomass conversion to alcohol. Difficulties at a pretreatment stage limit bioethanol production from biomass and do not allow to make this process economically viable [1, 14]. Further research is therefore needed to advance this technology until it becomes suitable for the consumer market.
Another potentially promising research area concerns combined finger millet and sweet sorghum (Sorghum saccharatum) biomass conversion described in a study [101]. As the first step of the investigation, the authors conducted a morphological analysis of finger millet and sorghum stems via microscopy to identify cell wall width. It was established that E. coracana has much thicker cell walls compared with sweet sorghum. The above-mentioned variety Tropikanka had at least 3 times thicker cell walls in vascular tissue than sweet sorghum had. This can probably refer to a higher content of lignin in millet straw. Such morphological analysis helped the authors to optimise the pre-treatment conditions. It was also identified that finger millet straw possessed higher hemicellulose content than S. saccharatum, while sweet sorghum, by contrast, had a higher level of cellulose [101].
Following the biomass composition studies, a technological protocol for bioethanol production from mixed finger millet and sweet sorghum raw materials was developed [101]. The pre-treatment process should not be very fast but ‘soft’ enough to avoid the formation of furfural (source for conversion are pentoses) and hydroxyl methyl furfural (from hexoses). These compounds are reported to cause a negative effect on microorganisms during fermentation, which can lead to the reduction of ethanol output [102].
The first proposed step in the technology is to obtain sorghum juice as a rich source of sugars. The juice was then condensed through evaporation and subsequently fermented. The leftover of juice pressing (bagasse) was mixed with ground finger millet biomass for thermal treatment at 90-95°C under acidic conditions (pH=3-4). It was proposed to use by-products of ethanol distillation and dehydration (referring to ethanol produced from sorghum juice). These steps were needed to reduce the amount of lignin and hemicellulose [101]. After that, cellulose was enzymatically treated using commercial enzymatic complex ACCELERASETM 1500 (Genencor). The authors also proposed to mix the obtained hydrolysate with fresh sorghum juice to facilitate further fermentation. The full scheme of the proposed bioethanol production from finger millet and sweet sorghum is shown in Fig. (5).
In addition, Tsygankov et al. [101] studied the monosaccharides composition in finger millet and sweet sorghum straw (air-dried biomass). The sugar composition of plant material was established by gas chromatography, which enabled to identify not only soluble sugars fraction, but also sugars from polysaccharides that are not available for hydrolysis (Fig. 6). It was shown that finger millet straw contained less glucose than sorghum bagasse (5.77% difference). At the same time, higher xylose content was identified in E. coracana biomass – up to 28.38%, while for sorghum bagasse, it was only 21.22%. The content of arabinose was also significant: 4.98-6.98%. These results indicate that finger millet straw may have a lower amount of sugars available for fermentation with S. cerevisiae.
After that, the content of cellulose and soluble sugars in dry biomass and the percentage of sugars available for fermentation (after enzymatic treatment) were investigated [101]. It was found that finger millet straw contained 35% wt. of soluble sugars and cellulose, while sweet sorghum bagasse had only 20% wt. For comparison, in this research, it has been established that sweet sorghum stems contained up to 40% wt. of soluble sugars and 35% (of dry biomass weight) of sugars, available for fermentation. Finger millet straw and bagasse samples contained (Fig. 5) only 10% wt. of sugar after enzymatic treatment that could be utilized by yeasts. Besides that, it has been shown that using the proposed technological scheme, it is possible to produce up to 4 m3 of ethanol from 1 t of bagasse and up to 6.6 m3 of ethanol from 1 t of E. coracana biomass [101].
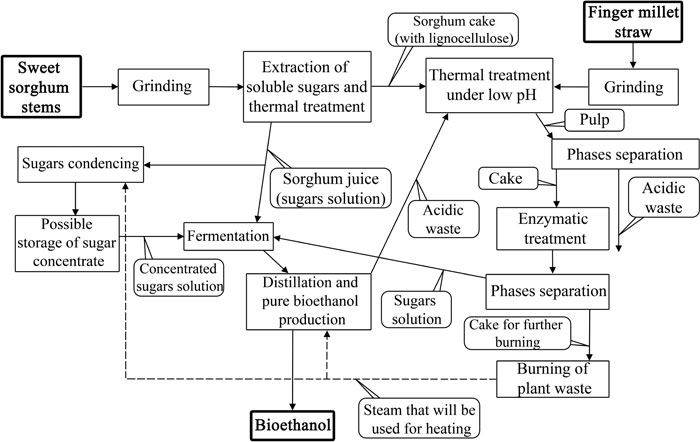
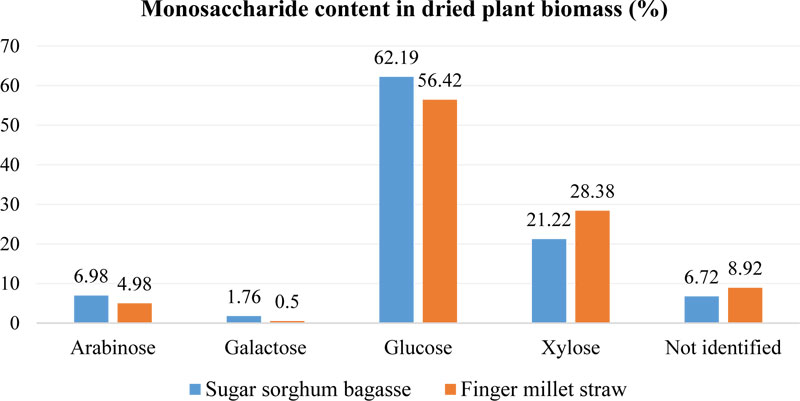
Processing Step | Feedstock | Conditions | Efficiency/Final Product Amount |
---|---|---|---|
Biomass pre-treatment | Dried, grinded straw (50g) | 100 mL of 0.5% sulfuric acid, heating up to 125-130°C under 25 psi pressure for 1 h | Pre-treated biomass with reduced cellulose crystallinity and reduced lignin amount |
Hydrolysis | 10% w/v of pre-treated biomass | 2% v/v of H2SO4, at 35°C for 4 days | 79.04-82.01% w/w of reduced sugars in the final solution |
Fermentation | Hydrolysed finger millet biomass | 4 g/L of yeasts, at 32.5°C and pH 6 for 4 days. Specific gravity before fermentation –1.042 | 6.92-7.28% w/v of ethanol in the solution after fermentation |
Solely usage of finger millet straw for bioethanol production has been recently studied [103]. Dried and ground biomass was pre-treated by adding 100 mL of 0.5% sulfuric acid to 50 g of sample (to remove lignin, reduce cellulose crystallinity) and heating it up to 125-130°C under 25 psi pressure for 1 h. The following step included a series of experiments to optimise the hydrolysis conditions. It was established that the best concentration of pre-treated biomass was 10% w/v of hydrolysis solution that contained 2% (v/v) of H2SO4, the pre-treatment hydrolysis lasted for 2 days at 30°C [103].
The other batch of experiments was focused on optimising H2SO4 hydrolysis. It was confirmed that the best concentration of H2SO4 was 2% v/v (that was used in the previous experiment) due to the highest measured content of sugars in the ultimate solution. In that case, other parameters remained unchanged from the previous experiment. Then the effect of temperature on hydrolysis in the range from 25-45°C was tested [103]. The hydrolysis solution contained additionally 10% w/v of pre-treated biomass, 2% v/v of H2SO4 and was incubated for 2 days. Samples that were kept at 30°C and 40°C showed very similar efficiency, while the highest sugar content in their end solutions was indicated in the sample that was treated at 35°C. It was also noted that too prolonged incubation decreased the efficiency of reduced sugars production and could lead to the formation of unwanted components (furfural and hydroxyl methyl furfural) [102, 103], as it was mentioned above.
At the last step of hydrolysis optimization, the effect of different incubation time in the range of 1-5 days was tested. All other conditions were kept unchanged as they were of the sample with the highest efficiency of sugars conversion. The optimal incubation time determined was 4 days, which allowed to obtain up to 79.04-82.01% w/w of reduced sugars (varied due to different methods of sugar content measurement). A full description of the most efficient conditions for this biomass processing step is provided in Table 3.
On the whole, the fermentation conditions have been optimised to make bioethanol production from finger millet more efficient [103]. The final product was ethanol, the concentration of which was expressed in % w/v. The effect of different pH levels on the fermentation has also been studied. For this purpose, authors used the following conditions: temperature - 30°C, yeast (S. cerevisiae) concentration - 4 g/L, fermentation time – 3 days, pH values varied from 4.0 to 6.5. The peak of the highest ethanol yield (6.08-6.58% w/v) was identified at pH 6. At higher pH levels, ethanol production started to decrease, while at pH 5.5, the difference in final C2H5OH concentration was not significant (5.48-5.97%). In further experiments, the authors kept the pH of the fermentation substrate at the optimal level (pH 6). At the next stage of optimisation, it was confirmed that for ethanol production from the finger millet-derived substrate, the optimal yeast concentration was 4 g/L.
Further studies of the effect of various temperatures on bioethanol production from finger millet revealed that the best optimal temperature was 32.5°C, which resulted in obtaining up to 7.12% w/v of ethanol (if substrate fermented at pH 6 for 3 days using 4 g/L of yeasts).
The last tested parameter for the optimisation of bioethanol production was fermentation time [103]. The authors identified that the optimal time for fermentation of processed biomass of finger millet was 4 days. These conditions allowed to produce up to 7.28% w/v of ethanol (Table 3). This ethanol production output was considered as high enough, comparing with acidic-hydrolyzed groundnut hulls (6.2%) and rice husks (5.5%) [103, 104]. In addition, the concentration of various metals (Cr, Fe, Mg, Pb, Ca) was identified in finger millet straw and produced ethanol from it. According to the obtained results, the authors concluded that the produced bioethanol was suitable for internal combustion engines [103].
Recently, the possibility of fermentation of finger millet straw using white-rot fungus (Trametes versicolor) instead of S. cerevisiae has been reported [105]. T. versicolor is known for its ability to utilize well both 5- and 6-carbon sugars, which makes it a very desirable biocatalyst for the conversion of plant biomass into bioethanol [106]. Another attractive benefit of T. versicolor is its high tolerance to most common fermentation inhibitors such as levulinic acid, hydroxyl methyl furfural, fural and phenols, which are consumed by this fungus [107]. For their study, the authors chose the acidic pre-treatment method of biomass samples [105]. Firstly, to prepare 10 g of hydrolysate, dried and powdered plant material was dissolved in 80 mL of 1% sulfuric acid and then autoclaved for 20 min (121°C, 1.8 bar). Secondly, this hydrolysate was filtered, the liquid was adjusted to pH 10, kept overnight, adjusted to pH 6 and cleared from the precipitate. That was done for rice paddy and biomass samples of finger millet, sweet sorghum and sugarcane (bagasse). Thirdly, the content of different monosaccharides in produced hydrolysates was studied before and after fermentation [105]. The total content of sugars in finger millet biomass hydrolysate was 8.29 g/L, which exceeded such value for sugar cane bagasse (7.09 g/L) but was lower than in the produced rice paddy (14.06 g/L) and sorghum straw (10.05 g/L) hydrolysates (Fig. 7).
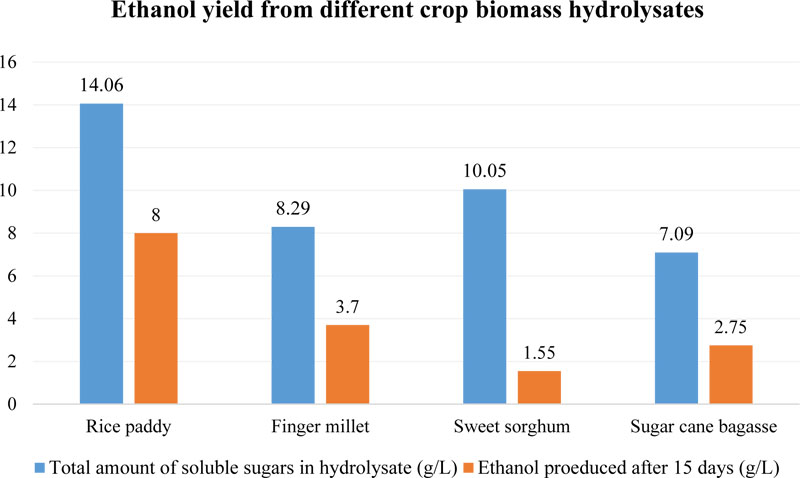
At the same time, finger millet biomass hydrolysate contained 56.7% of xylose, which was in a significantly higher amount than it was identified in a previous study [101] for its dried unprocessed straw (28.38%). The glucose content was much lower in the hydrolysate of E. coracana (22.9%), than in the untreated biomass (56.42%). In addition, it was established that among other studied feedstocks, finger millet possessed the second highest content of glucose after rice paddy (42.7%) [105].
More interesting results were obtained after fermentation tests with T. versicolor, which revealed that that fungus was able to utilize up to 99% of sugars from finger millet hydrolysate in 15 days, while in the case of processed rice paddy, the sugar consumption rate was only 90% and for sweet sorghum - 85%. Besides that, rice paddy hydrolysate allowed to produce the highest amount of ethanol (up to 8 g/L), probably, due to the highest content of soluble sugars [105]. Finger millet sample was the second most efficient feedstock with 3.7 g/L content of ethyl alcohol. It is interesting to note that E. coracana allowed obtaining at least twice the amount of ethanol comparing with sweet sorghum (1.55 g/L), which contained much more soluble sugars (10.05 g/L) than finger millet (8.29 g/L) in their hydrolysates (Fig. 7).
The next research objective was to study activity changes of two T. versicolor enzymes (acetaldehyde dehydrogenase and pyruvate decarboxylase) during fermentation. Acetaldehyde dehydrogenase activity reached its peak on the third day of fermentation. This enzyme is known to convert acetaldehyde to acetate, which has a negative impact on ethanol production efficiency [105]. After the third day and during the rest of the fermentation time, acetaldehyde dehydrogenase activity decreased, while another enzyme, pyruvate decarboxylase, showed increasing activity as fermentation progressed and reached its maximum on the fifteenth day. This enzyme enables non-oxidative decarboxylation of pyruvate to acetaldehyde, thus providing more substrate for further conversion into ethanol. These processes are well studied for S. cerevisiae [108], unlike for T. versicolor. These findings are very important to further optimise the fermentation conditions and to improve the bioethanol production approach. T. versicolor fermentation of plant biomass, especially of agricultural waste like finger millet straw, seems to be very promising, but the main disadvantage of the technology that could limit its industrial implementation is a long fermentation time – up to 15 days, while S. cerevisiae needs only 4 days for efficient ethanol production, which is shown above [103], (Table 3).
There are also other reports on research devoted to bioethanol production from E. coracana biomass [109]. The research was focused on the utilization of finger millet husk, which was usually a by-product generated during harvesting of this crop. Four different husk samples of different local varieties were collected for the study. Before biomass pre-treatment, contents of reducing sugars, total sugars and cellulose were estimated. This analysis was used to identify the best biomass feedstock (with the highest amount of reducing sugars and the lowest content of cellulose) for further bioethanol production experiments.
After that, the authors [109] tested various biomass (husk) pre-treatment approaches: physical, acidic and alkaline methods [110]. The enzymatic analysis was performed with Cellulomonas fumi (NCIM 5015), which is known as a producer of cellulase enzymes. These conditions with detailed descriptions are provided in Table 4. The authors also conducted FTIR analysis to confirm changes that happened during pre-treatment (i.e., decrease in lignin peak). After that, the authors followed the SSF protocol [111]. Fermentation was conducted using S. cerevisiae isolated from muskmelon. As many details of pre-treatment and fermentation were not specified [109], this report gives only a general overview of possible approaches for finger millet husk utilization.
It was revealed that the most effective approach of bioethanol production from finger millet husk was the procedure that involved enzymatic treatment, which allowed to produce up to 5.1% of ethanol [109]. The second most efficient approach was based on pre-treatment under acidic conditions (3.9% of ethanol). Thus, the use of C. fumi for hydrolysation of finger millet husk allowed increasing ethanol yield approximately by 30%, compared to acid- or alkali-hydrolysed samples. Nevertheless, the authors [109] have not given temperature conditions or duration of pre-treatment and fermentation stages, so it is impossible to conclude, if the reported ethanol production rates were the highest possible, or if the production conditions could be further optimised for higher efficiency (as it was previously done [103]).
The possibility of enzymatic saccharification of finger millet biomass was studied previously [112]. The authors tested the efficiency of recombinant enzymes on biomass that had been pre-treated in different ways. In their research, endo-1,4-β-xylanase (CtXyn11A, from Clostridium thermocellum) and exo-1,4-β-xylosidase (BoGH43A, from Bacteroides ovatus) were used, which carry out the conversion of xylan to xylose, thus reducing hemicellulose. It was established that the best saccharification results could be obtained by using endo-1,4-β-xylanase on finger millet straw pre-treated with 1% w/v NaOH for 20 min at 120°C [112]. This enabled to increase the content of reducing sugars and holocellulose (from 69% to 76%), which was confirmed by FTIR and HPLC analyses. The authors also conducted the optimization of hemicellulose saccharification conditions for these enzymes, which revealed that maximal efficiency could be reached at pH 7.5 and at 55°C and 37°C for endo-1,4-β-xylanase and exo-1,4-β-xylosidase respectively. The percentage of conversion of xylan to xylose was 24.7% by both enzymes [112].
The use of various cell wall degrading or fibrolytic enzymes has great potential that can be applied in the production of the second-generation bioethanol. Within that, each biomass feedstock required individually optimized pre-treatment approaches [113], therefore the search of more active bacterial enzymes could help to resolve this issue. It was also reported about the metagenomics study of rumen microflora of Indian cattle that were fed with finger millet straw only. The authors reported that a very diverse group of glycoside hydrolyses was identified [114]. These findings may refer to the ability of some of such enzymes to degrade lignocellulose-rich plant biomass. The main genera, contributing carbohydrate-active enzymes (CAZ), are Prevotella, Bacteroides, Fibrobacter, Clostridium and Ruminococcus. Usage of CAZ, produced by rumen resident microbiota, could provide opportunities for significant improvement of the second-generation bioethanol production from cellulosic feedstock, especially finger millet biomass.
4. TECHNO-ECONOMIC ANALYSIS OF BIOETHANOL PRODUCTION FROM FINGER MILLET
Economic aspects of bioethanol production from both finger millet grain and straw remain almost unstudied. Although the technology for finger millet agricultural products conversion is sufficiently developed, no complex assessments on its economic effect have been conducted yet. In general, almost all starch-based bioethanol production life-cycles are being evaluated as potentially feasible [115], but, in this case, the competition between food and industrial grain utilization may arise [1]. From this point of view, ethanol production from straw is being an attractive alternative to starchy feedstocks, since this type of biomass is usually considered as agricultural waste. However, probably the most crucial bottleneck for the implementation of these bioethanol-producing technologies is a volatile price for fossil oil, which may have a negative impact on biofuels production profitability.
Besides that, the feasibility of the conversion of straw (from various plant sources) into bioethanol was shown earlier, even compared with fossil fuels [115]. Additionally, in the mentioned review [115], it was discussed that GHG emissions, in this case, could be reduced by 76%. Sustainability of straw conversion was shown for rice [116, 117], wheat [118], barley [119], sweet sorghum [120, 121] and pearl millet [122] as it took place in a wide range of European, Asian and North American countries. These results provide evidences that the application of bioethanol production, based on finger millet, might also be successful and economically feasible. As it was mentioned above, finger millet is able to grow on marginal lands that are not suitable for other crops cultivation [25, 52, 53]. This suggests that the cultivation of E. coracana on such territories for bioethanol production purposes may be reasonable and would avoid competition with other traditional food crops.
Pre-treatment or Hydrolysis Method | Conditions | Efficiency/Final Product Amount |
---|---|---|
Physical | Dried, chopped husk was ground until homogenous powder obtained | 3.0% of ethanol |
Acidic | Powdered biomass, 2% H2SO4, other not specified [110] | 3.9% of ethanol |
Alkaline | Powdered biomass, 3% NaOH, other not specified [110] | 3.6% of ethanol |
Enzymatic | Powdered biomass, Cellulomonas fumi pre-cultured on LB, added in an unknown amount | 5.1% of ethanol |
Another proposed approach is based on the idea to combine first- and second-generation bioethanol production [123]. The mentioned study describes the consolidation of both generation processes to reduce the production cost of first-generation ethanol by integrating straw-derived alcohol utilization for energy needs for ethanol obtaining from grain [123]. Theoretically, such an approach may be applicable also for finger millet, especially, in the countries, where this crop is not traditionally used for food purposes. On the other hand, the same approach could be potentially applied for non-industrial finger millet cultivation. In this case, straw or agricultural waste, such as husk, could become a feedstock for bioethanol production, which could cover part of the energy needs for crop growing. However, such approaches require additional studies to prove their feasibility.
As it was mentioned previously, finger millet is the most widely cultivated crop in African and Asian (especially in India) countries [28]. In the FAO report on millets [124], it was mentioned that finger millet was considered as a low-risk crop due to its high abiotic stress tolerance that results in a relatively stable grain harvest, independently from unfavourable weather or climate conditions. In general, the cultivation of finger millet was shown to be profitable in different regions [125-127], which could suggest that the conversion of straw or agricultural waste would enhance the profitability of the cultivation of this crop. Moreover, as it was discussed above, finger millet possesses great potential for improvement, especially using novel biotechnology tools [27]. Additionally, genetic engineering is expected to be one of the most effective instruments that could help to increase the productivity of cellulosic feedstocks [128], including finger millet, that in the future will have a positive impact on the feasibility of the second-generation bioethanol production. Besides that, a huge number of investigations on various aspects of second-generation bioethanol obtaining are required (e.g., biomass pre-treatment, search for new yeast strains, etc.) to optimize this process and, thus, increase profitability [128]. As it has been discussed in this review, research on the possibility of bioethanol production from finger millet has just started and has already provided many interesting technological solutions for its improvement. Nevertheless, this topic needs to be much more developed, before it could be applied.
CONCLUSION AND FUTURE PROSPECTS
Finger millet (E. coracana) is a very promising alternative crop that can be used not only for the production of high nutritionally valuable food, but also as an efficient source of biomass for bioethanol production. This millet is very attractive for utilization as a bioenergy crop due to several advantages that, when realized in the future, can further improve this crop. From this point of view, the creation of new highly biomass-productive genotypes using molecular marker-assisted breeding based on the whole genome sequencing data looks very promising. In addition, genetic engineering protocols are already developed for finger millet that can significantly simplify the obtaining of plants with new agronomic valuable traits. Biomass productivity of finger millet can also be increased by using non-transgenic methods, such as induction of genome instability. This may foster manifestation of useful mutations, similar to those prompted with somaclonal variability.
Seed and green biomass productivity of this crop could be further improved to raise the efficiency and economic viability of bioethanol production. Thus, previous studies reported about the possibility to obtain up to 3800 L of ethanol from 9.85 t of E. coracana seed yield from 1 ha using standard fermentation techniques. Other research showed the possibility to apply VHG fermentation techniques to finger millet as a starchy feedstock. Utilization of different yeasts as biocatalyst for ethanol conversion was shown for the ragi flour-based VHG approach, which results in 13.1% of alcohol yield in the final product of fermentation. Besides that, malted finger millet can be a valuable source of very active α- and β-amylases that may be used as an additive to other starch feedstocks to increase the efficiency of their saccharification.
Biomass conversion into bioethanol requires more technological steps, which all should be optimized specifically for each separate feedstock. At present, there are technological procedures for joint finger millet and sweet sorghum conversion to bioethanol that have been adjusted for industrial scales production of up to 6.6 m3 of ethanol from 1 t of E. coracana biomass. There are also other reports stating the development of a protocol for finger millet straw conversion via both acidic pre-treatment and hydrolysis for further fermentation with S. cerevisiae. It was shown that this approach allowed obtaining a fermentation product containing up to 7.28% of ethanol. Such a productivity rate is comparable with other agricultural waste feedstocks (e.g., rice husks).
The possibility of the usage of other organisms as biocatalysts instead of yeast has also been studied. White-rot fungus (T. versicolor) was shown as a good alternative to S. cerevisiae in the conversion of finger millet straw, since this fungus is able to utilize both pentoses and hexoses. It has been shown that T. versicolor was able to utilize up to 99% of soluble sugars, which was the highest rate comparing to other common bioethanol feedstocks (rice paddy, sweet sorghum, sugar cane bagasse). In addition, a great perspective is held by various methods of enzymatic hydrolysis of plant biomass, including E. coracana. It has been reported that the use of C. fumi for hydrolysation of finger millet husk allowed to increase ethanol yield approximately by 30%, compared with acid- or alkali-hydrolysed samples. Recently, the possibility of finger millet straw saccharification with recombinant enzymes has been successfully tested. Usage of endo-1,4-β-xylanase (CtXyn11A) and exo-1,4-β-xylosidase (BoGH43A) made it possible to convert up to 24.7% of xylan to xylose, thus reducing the content of hemicellulose in finger millet straw. This finding may also be applied in industrial bioethanol production, increasing the efficiency of usage of E. coracana as cellulosic feedstock.
However, as many other technologies for second-generation bioethanol production, finger millet biomass conversion requires further improvement to increase productivity and profitability. No research has been done yet to shed light on the economic viability of biofuel production from E. coracana. Furthermore, not all options were exploited for the improvement of existing ethanol-production technologies based on finger millet. Such approaches as VHG fermentation could be applied not only for starchy feedstocks, but also for treated biomass, including E. coracana. Very limited research has been done on the fermentation of finger-millet based substrates using different species of microorganisms or using specially bred yeast strains. This proves to be a huge field of study, taking into account that optimization of production technologies (various pre-treatment approaches, saccharification) is required for fermenting organisms. In addition, search for new more efficient enzymes with cellulose degrading activity is extremely important for better finger millet biomass saccharification and more efficient bioethanol production. Similarly to the development of ethanol production methods, the improvement of finger millet’s biomass productivity is equally important. The wide spectrum of currently available novel molecular genetics approaches opens new horizons for the enhancement of this crop.
CONSENT FOR PUBLICATION
Not applicable.
FUNDING
None.
CONFLICT OF INTEREST
The authors declare no conflict of interest, financial or otherwise.
ACKNOWLEDGEMENTS
We acknowledge the suggestions by Dr. V. Kyrylenko (Institute of Food Biotechnology and Genomics, National Academy of Sciences of Ukraine, Kyiv), aimed to improve the manuscript language.