All published articles of this journal are available on ScienceDirect.
Helminthosporiosis Impact on the Photosynthetic Apparatus and the Oxydative Status of Barley Seedlings at Different Stages of Development
Abstract
Background:
In recent years, spot blotch, a disease caused by Bipolaris sorokiniana (Sacc.) Shoem., has emerged as a serious concern with regard to spring barley cultivation. However, the effect of this pathogen on the photosynthetic apparatus and oxidative status of barley plants at different stages of chloroplast development has hardly been studied.
Aims / Methods:
The study aimed to evaluate the photosynthetic apparatus and protective system of a spring barley’s (Hordeum vulgare L.) response to Bipolaris sorokiniana (Sacc.) Shoem. (B.S.) at different stages of chloroplast biogenesis. The green seedlings of different ages (3-11 days) were treated with fungal spores (106 spores×ml−l). The first leaves of seedlings were analyzed 24 or 48 hours after the inoculation with the pathogen.
Results:
Differences in response to fungal infection were identified in leaf tissues containing chloroplasts at different stages of development. The differences manifested themselves in the non-photochemical quenching (NPQ) of absorbed energy, the total content of reactive oxygen species (ROS), lipid peroxidation (LPO) activity and polyphenol content. An increase in peroxidase (POX) activity in infected barley seedlings at the anabolic stage of chloroplast biogenesis and its decrease at the catabolic stage against the background of a constant increase in ascorbate peroxidase (APX) activity were revealed. A high positive correlation between the chlorophyll and malondialdehyde content (MDA) was observed.
Conclusion:
In barley seedlings of different ages, a response to fungal contamination is different in the PS II activity, the general content of ROS and polyphenols, as well as the LPO activity. The photosynthetic and oxidative parameters of barley plants may be a useful tool to control plant immunity in view of breeding and novel protective technologies.
1. INTRODUCTION
A level of cereal yields and their quality largely depend not only on a crop management system and the genetic potential of a given cultivar, but also on the factors that may cause damage to plants or reduce yields. Such factors include fungal diseases of cereals that may cause a reduction in yields by 15-20% and, in extreme cases, even by 60% [1].
Barley (Hordeum vulgare L.), one of the world’s oldest cultivated crops, is currently the fourth most important cereal in Belarus and globally. Spot blotch caused by Bipolaris sorokiniana (Sacc. in Sorok.) Shoem. has emerged as a serious concern for barley cultivation, reducing its yields, as well as the quality of barley grain. It has been shown that high temperatures and high relative humidity are most favorable for the outbreak of this pathogen. Thus, spot blotch is considered an increasing threat to barley cultivation under a changing climate [2].
In the course of evolution, plants have developed protective systems against a wide range of pathogens [3] with membrane receptors that recognize particular molecular motifs of a pathogen referred to as pathogen-associated (or microbe-associated [4, 5]) molecular patterns (PAMP/MAMP) considered as central elements [6]. This recognition quickly initiates subsequent events; among them are the activation of signal pathways, transcription reprogramming and a protective response [7]. In addition, it is important to mention interactions between the signaling pathways of biotic and abiotic origin, circadian rhythms, photoperiodic and hormonal systems. As a result, cross signals may occur, leading to both synergistic and antagonistic responses that are difficult to predict.
The study of plant protective reaction mechanisms in pathogenesis inevitably leads to the clarification of the role of both light as a fundamental regulatory factor and chloroplasts as sensors that subtly respond to external stimuli. A special role of plastids in the immune system is also associated with their participation in the biosynthesis of several types of key protective molecules, including hormones (salicylate, jasmonate, and abscisic acid) and secondary messengers such as calcium, ROS [8], which activate mitogen-activated protein kinases (MAPKs) [9]. It is rather obvious that the study, addressing the role of plastids in the immune system mainly focuses on the problem covering the interaction between the chloroplasts and the plant pro- and antioxidant systems [10].
While communication mechanisms between the organelles and the different cell compartments in terms of ROS seemed disappointingly complex until quite recently [11, 12], progress in this field is evident. Thus, it has been shown that light-induced ROS, which was generated by electron flow in chloroplasts, mediates retrograde signals involved in the regulation of the expression of protective genes in the nucleus [13-15]. At the same time, it has been found that the ROS produced by the redox system of chloroplasts are key participants in signaling pathways under various types of stress [16-23].
To better understand the role that light plays in the mechanism of a plant immune response, it is necessary to systematically investigate changes occurring in the content of both the ROS and a number of other parameters of oxidative status, depending on the structural and functional state of plastids when infected with the pathogen.
It is well known that the seedlings of different ages are characterized by various rates of photosynthetic reactions. Photosynthetic activity decreases with leaf aging [24-26], which is conditioned by the destruction of an electron transport chain in chloroplasts and the inactivation of Calvin cycle enzymes. Therefore, it is possible to suppose that seedlings of different ages could possess variable stability under stress conditions, including pathogenesis.
In the course of this work, we used the seedlings of spring barley Hordeum vulgare L. developed in the light that contained chloroplasts possessing different amounts of photosynthetic pigments and being characterized by photosynthetic activity. The pathogenic fungus B.S. was applied as an infecting agent causing dark-brown spotting [27]; the infection was carried out in the light.
The aim of this research was to evaluate the photosynthetic apparatus and oxidative state of a spring barley response to fungus B.S. at different stages of chloroplast biogenesis, significantly contributing to the development of representations about the role of chloroplasts in the formation of an immune response in the plant cell.
2. MATERIALS AND METHODS
2.1. Plant Material and Growth Conditions
Experiments were carried out using the green seedlings of spring barley of the Magutny variety. Green seedlings were grown in a climate chamber under the conditions of polychromatic white light (120 μmol quanta m−2s−1) with a photoperiode of 16 h light/8 hours of darkness at a temperature of 22°C. The intact green barley seedlings of different ages (3-11 days) were treated with a suspension containing the spores of the fungal pathogen B.S. (106 spores×ml−1) in the light. The effect of fungal contamination was evaluated 24 or 48 hours after the inoculation of green seedlings. The plants treated with distilled water served as the negative control.
2.2. Estimation of Photosynthetic Parameters
Chlorophyll (Chl) pigments were analyzed after the extraction with acetone [28].
The carotenoids were determined by high-performance liquid chromatography (HPLC) using the Shimadzu LC20 Prominence device equipped with NUCLEODUR C18 Gravity columns (3×150 mm, Macherey Nagel, Germany) [29]. Pigments were detected by the spectrophotometric detector with the SPD-M20A Prominence diod matrix (Shimadzu, Japan), applying absorption spectra in the range of 200-700 nm.
Photochemical photosystem II (PS II) activity, the most sensitive component of the photosynthetic apparatus, was assessed using the pulse amplitude modulated (PAM) fluorometry applying the Dual-PAM 100 fluorometer (Walz, Germany) [30].
2.3. Estimation of Oxidative State Parameters
The LPO activity was assessed by the amount of MDA detected by the optical density of its reaction product with thiobarbituric acid at 532 nm [31].
The overall level of ROS was determined in leaf extracts using a fluorescent test relying on the formation of 2´,7´-dichlorfluorescein (DCF) from non-fluorescent dichlorfluorescein diacetate (DCFDA) [32]. Fluorescence of DCF (λex=496, λem=526) was measured using the SOLAR SM 2203 spectrofluorometer (SOLAR, Belarus) [33]. It should be kept in mind that while H2O2 and several lipid hydroperoxides are reported to oxidize DCFDA, it results in the ability of other ROS, such as superoxide anion (O2•-) and hydroxyl radical (•OH) stimulate the formation of DCF, are inconclusive [32].
2.4. Antioxidant Enzymes Activity
Peroxidase (POX, EC 1.11.1.7) activity was assessed spectrophotometrically using the Shimadzu UV-2401PC device (Shimadzu, Japan) [34] with benzidine as a substrate. Its peroxidase-mediated oxidation in the presence of hydrogen peroxide gives a compound possessing an absorption maximum at 605 nm, and the period of time required to reach the maximal optical density is measured. To determine the protein content in the sample, the Lowry protein assay [35] was used.
Ascorbate peroxidase (APX, EC 1.11.1.11) was assayed by recording a decrease in the optical density due to ascorbic acid at 290 nm. APX catalyzes the decomposition of hydrogen peroxide in the presence of ascorbic acid towards water and monodehydroascorbate [36].
2.5. Polyphenols Content
The total number of polyphenols was determined spectrophotometrically using the Folin-Denis method [37]. Along with the course of oxidation with tungstic acid under basic conditions, the absorption was measured at 765 nm. Gallic acid was applied as a calibration standard.
2.6. Statistics
The results are the mean of three biological and three experimеntal replicates of each experiment. The results are presented as a mean ± standard error (SE). The data were statistically analyzed by the one-way analysis of variance (ANOVA), and mean separations were compared with the Student-test using “Statistica 6.0” (StatSoft) and “Excel 2010” programs. Differences at p ≤ 0.05 were considered to be significant and are marked on the graphs and tables with an asterisk.
3. RESULTS
Fig. (1) exemplarily illustrates a change in the content of photosynthetic pigments along with the development of barley seedlings of Magutny variety (see Methods). It is clearly seen that starting from a certain age, the Chl content in the infected tissues of green plants decreased compared to the control group. At the same time, the carotenoids content decreased significantly during infection in 13-day-old seedlings only.
The results of the chromatographic separation of pigments of the xanthophyll cycle are presented in Table 1. As can be seen from the data obtained, the content of xanthophyll pigments decreased both in healthy and infected leaves as a result of aging. Against the background of a decrease in the total amount of xanthophyll pigments in ontogenesis and upon infection (starting from 6 days of age), no significant changes were revealed in the ratio of carotenoids within the xanthophyll cycle that usually responds to a number of abiotic stressors.
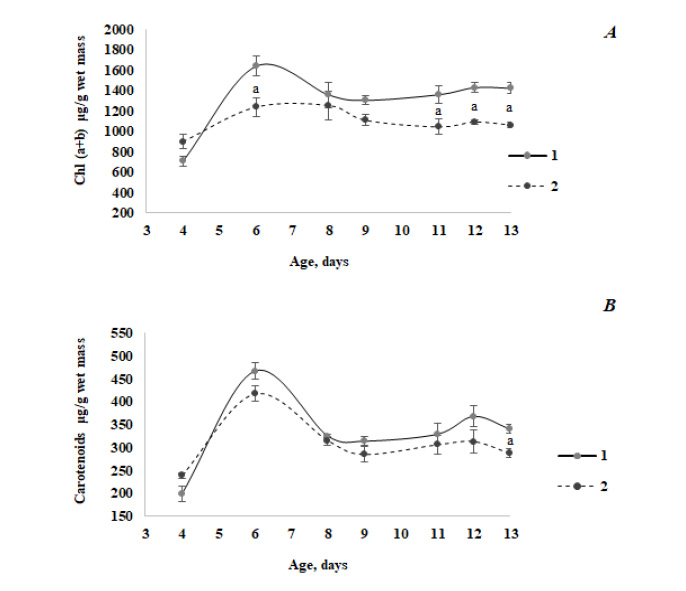
The study of the photochemical activity of stress-sensitive PS II using PAM-fluorometry methods has revealed some peculiar features of its behavior both in the course of ontogenesis and in the case of infection. The NPQ of absorbed energy in green seedlings decreased in the ontogenesis of the control variant and increased in the case of infection (Fig. 2A). Thus, compared to the control, the pattern of the NPQ change in ontogenesis was reversed in infected seedlings. The photochemical quenching of absorbed energy (qP) (Fig. 2B) practically did not change in control, but in the aged seedlings, a noticeable inhibitory effect of fungal contamination was observed. At the same time, a relative decrease in the value of qP in the case of infection was slightly lower than a relative increase in NPQ.
Analysis of the total ROS content in the barley seedlings of different ages showed that in healthy plants, as they developed, this parameter naturally decreased and turned out to be 4.2 times lower than in 9-day-old seedlings relative to 5-day-old ones (Table 2). However, reaching the age of 12 days, the ROS content significantly increased again, which is associated with the onset of aging processes and the destruction of the photosynthetic apparatus when growing seedlings in water without mineral nutrition. The same tendency was found for infected barley seedlings, although with a less pronounced amplitude of changes in the ROS content during plant development. Therefore, the total ROS content changed during plant development but always remained higher under fungal contamination (Table 2).
As a result of the interaction of oxidant and antioxidant systems, the LPO activity in the young seedlings of the control variant was higher than in the aged ones, but at all stages of ontogenesis, its value under the fungal infection was lower than in control (Table 3). This finding suggests that the trends of changes in the LPO activity and the total ROS content in the ontogenesis of green seedlings were opposite. It can be seen that in the case of infection, peroxidase activity reliably exceeded the activity observed in the control only in the seedlings that had finished their growth and possessed mature chloroplasts. In aging leaves, peroxidase activity in the case of infection decreased compared to the younger ones, containing mature plastids. Infection had a stimulating effect (at least during the experiment, i.e., 2 days after the inoculation) on the activity of ascorbate peroxidase reaction, the central transformation within the cycle aimed at the removal of the main ROS in chloroplasts. APX activity in infected leaves was significantly higher compared to the control.
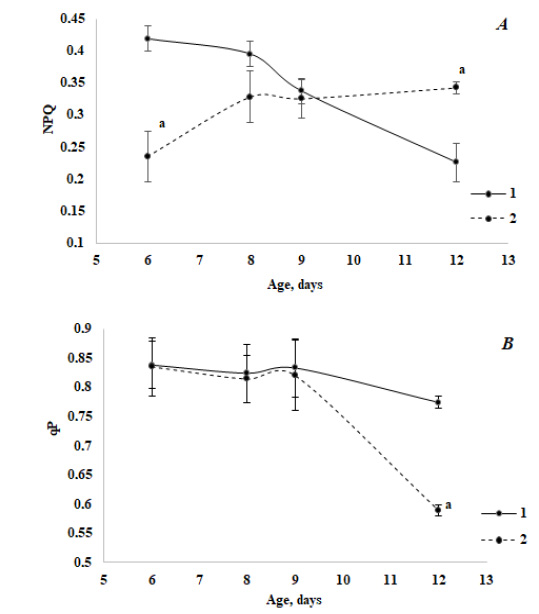
a Differences are significant between the control and B.S. (t-test, P≤0.05).
Variant | Pigment, % | ΣXanthophylls,μg g−1wet mass | |||
---|---|---|---|---|---|
Age at analysis, days | Treatment | Violaxanthin | Antheraxanthin | Zeaxanthin | |
5 | Control | 59.8 | 40.2 | − | 82.8±1.0 |
B.S. (48 h) | 58.6 | 41.4 | − | 91.2±0.5a | |
6 | Control | 62.8 | 40.9 | 16.3 | 82.8±1.4 |
B.S. (24 h) | 65.9 | 44.2 | 17.9 | 62.4±2.4aс | |
8 | Control | 32.8 | 46.0 | 21.2 | 52.8±1.2b |
B.S. (24 h) | 30.2 | 43.9 | 26.0 | 28.5±0.5ac | |
9 | Control | 34.7 | 46.3 | 19.0 | 45.8±0.9b |
B.S. (48 h) | 37.2 | 48.7 | 14.1 | 30.4±2.2ac |
b Differences are significant between the 5-day-old healthy seedlings and the older ones (t-test, P≤0.05).
c Differences are significant between the 5-day-old infected seedlings and the older ones (t-test, P≤0.05).
Variant | ROS content | ||
---|---|---|---|
Age at analysis, days | Treatment | mg DHF·g-1 wet mass | % to Control |
5 | Control | 2.23±0.10 | 100 |
B.S. (48 h) | 2.86±0.14a | 128 | |
7 | Control | 2.02±0.01 | 100 |
B.S. (48 h) | 2.73±0.01a | 135 | |
8 | Control | 0.92±0.03b | 100 |
B.S. (24 h) | 1.53±0.10ac | 165 | |
9 | Control | 0.53±0.08b | 100 |
B.S. (48 h) | 1.11±0.05ac | 187 | |
12 | Control | 1.65±0.10b | 100 |
B.S. (24 h) | 2.47±0.20a | 150 |
b Differences are significant between the 5-day-old healthy seedlings and the older ones (t-test, P≤0.05).
c Differences are significant between the 5-day old infected seedlings and the older ones (t-test, P≤0.05).
Variant | MDA content | ||
---|---|---|---|
Age at analysis, days |
Treatment | nmol/g wet mass | % to Control |
6 | Сontrol | 141.8±5.0 | 100 |
B.S. (48 h) | 86.6±4.0a | 61 | |
8 | Сontrol | 117.6±2.4 b | 100 |
B.S. (24 h) | 109.6±2.0bc | 93 | |
9 | Сontrol | 118.8±3.6b | 100 |
B.S. (48 h) | 102.6±2.4ac | 86 | |
12 | Сontrol | 118.0±2.8b | 100 |
B.S. (24 h) | 103.0±2.4ac | 87 |
b Differences are significant between the 6-day-old healthy seedlings and the older ones (t-test, P≤0.05).
c Differences are significant between the 6-day-old infected seedlings and the older ones (t-test, P≤0.05).
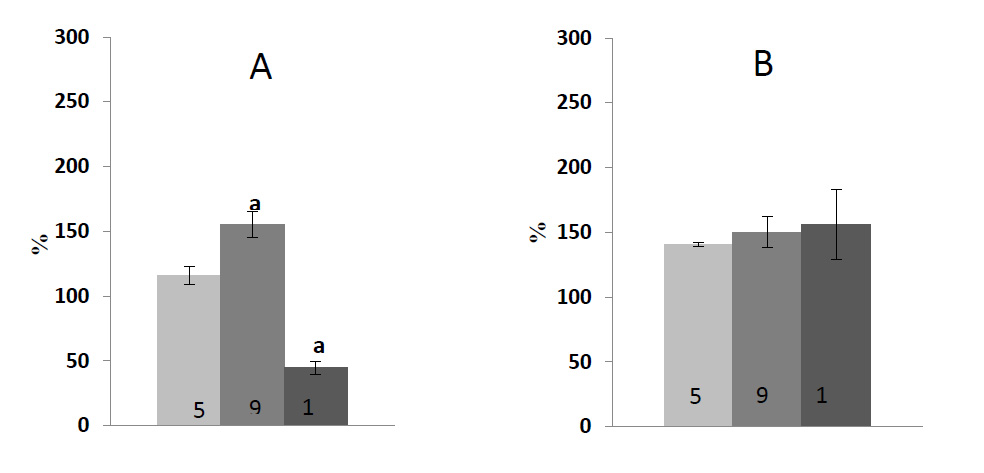
a Differences are significant between the 5-day-old seedlings and the older ones (t-test, P≤0.05).
Fig. (3) shows data on an ontogenetic series of experiments assessing the activity of two antioxidant-protecting enzymes. The experiments were performed using the same material. The maximum value of total protein in leaf tissue was found in 9-day-old seedlings. The fungal infection led to its decrease, which is especially noticeable upon the catabolic development (an established decrease ― more than 50%). In the case of anabolic development, the value was about 11-16% compared to the control (data not shown). It can be seen that in the case of infection, the POX activity reliably exceeded the activity observed in control only in the seedlings that had already finished their growth and possessed mature chloroplasts. In aging leaves, the POX activity decreased in the case of infection compared to the younger ones containing mature plastids. The infection had a stimulating effect (at least during the experiment, i.e., 2 days after the inoculation) on the APX reaction activity; the central transformation within the cycle aimed to remove the main ROS in chloroplasts. The APX activity in infected leaves was significantly higher compared to the control.
It is well documented that phenolic compounds, being secondary plant metabolites, perform antioxidant functions in plants under various stress conditions. In ontogenetic studies with green barley seedlings, it was found that the highest absolute content of polyphenols is characteristic of 12-day-old seedlings, and the most significant stimulation of their synthesis during the infection occurred in 9-day-old plants (Table 4), i.e., before the clear onset of the catabolic branch in the kinetics of Chl accumulation.
Variant | Polyphenols’ Content | ||
---|---|---|---|
Age at Analysis, Days |
Treatment | mg/ g Wet Mass | % to Control |
7 | Сontrol | 1,09±0,02 | 100 |
B.S. (24 h) | 1,17±0,05 | 107 | |
8 | Сontrol | 2,99±0,06b | 100 |
B.S. (24 h) | 3,00±0,00c | 100 | |
9 | Сontrol | 2,70±0,04b | 100 |
B.S. (24 h) | 4,24±0,07ac | 157 | |
12 | Сontrol | 6,54±0,03b | 100 |
B.S. (24 h) | 7,94±0,07ac | 121 |
b Differences are significant between the 7-day-old healthy seedlings and the older ones (t-test, P≤0.05).
c Differences are significant between the 7-day-old infected seedlings and the older ones (t-test, P≤0.05).
It is obvious that almost all the examined oxidative stress markers changed in ontogenesis when infected with a pathogen. This is also valid for the parameters of the structural and functional state of chloroplasts. Therefore, the cooperation between oxidative parameters (LPO, ROS content) and the functional state of the photosynthetic apparatus (Chl (a+b) and the carotenoid content, NPQ) was estimated. First of all, this is a high ratio of the positive correlation (r = +0.83) between a change in the Chl content (in a fairly wide range) and the LPO activity estimated by the MDA content. The level of LPO activity reflects the function of antioxidant systems aimed at protection from the oxidative stress caused by aggressive ROS. It is rather logical to assume that high LPO activity should be a consequence of a high ROS level in cases where antioxidant protection is insufficient. However, in our hands, changes in the overall ROS content demonstrated not only a minor correlation with the LPO activity observed in similar experiments, but also a negative trend (r = − 0.58). While the ROS content was well correlated with that of total carotenoids (r = + 0.86), a high positive correlation between changes in the NPQ value and the level of LPO activity (r = + 0.71) is rather interesting, though not entirely clear.
4. DISCUSSION
We have found that starting from the age of 6 days, the Chl and carotenoids’ content in the infected green leaves of barley seedlings decreased compared to the control group (Fig. 1), which is in good agreement with the published data [38, 39]. Thus, it was observed that silencing of carotenoid or Chl biosynthesis at phytoene desaturase or Mg-chetalase H steps, respectively, led to a faster appearance of hypersensitivity reaction (HR) symptoms during the infection of wheat with hemibiotrophic fungus Zymoseptoria tritici. In the rice leaf tissue 3 days after the infection with fungus Rhizoctonia solani, the structural disintegration of chloroplast (grana, thylakoid and stroma organization) was revealed [40]. Moreover, chloroplasts were the main source of ROS formation at that time, accompanied by the decreased photosynthetic performance.
The positive fungus effect on the Chl content was only noted in four-day-old green infected leaves (Fig. 1A). It seems counter-intuitive to observe positive effects on chloroplast functions during a hemitrophyc fungal attack, although this phenomenon has recently been demonstrated for Alternaria alternata fungal pathogen [41]. Volatile compounds of beneficial microorganisms are known to have positive effects on the plant growth and its photosynthesis capacity and work with A. alternata indicates that volatiles of necrotrophic fungi also have similar effects on plants [41]. Thus, PS II operating efficiency and photochemical quenching were enhanced, and the Chl content was increased in proximity to A. alternata volatiles [41]. A similar effect may be assumed in the case of infection with fungi, which manifested itself only in the leaves with poorly developed chloroplasts.
The xanthophyll cycle is known to be involved in the dissipation of excess light energy to protect the photosynthetic apparatus through the NPQ of chlorophyll fluorescence. Recent studies have demonstrated that NPQ may be positively or negatively affected by the pathogen attack at an early stage of infection [42]. The HPLC analysis showed that infection of Arabidopsis leaves with the necrotrophic pathogen Sclerotinia sclerotiorum caused the substantial quantities of zeaxanthin at the expense of violaxanthin with a corresponding decrease in the neoxanthin content. In our experiments, no changes in the amount of pigments of the xanthophyll cycle were found in the leaves infected with B.S. (Table 1). The resulting effect may be determined by the specificity of plant-pathogen interaction in this case.
It is known that Chl fluorescence represents a particularly fast method allowing to obtain detailed information about PS II photochemical activity, electron transport events and different regulatory processes. Recently, the Chl fluorescence measurement has gained popularity as a technique for identifying stress in plants [43]. In the course of green seedlings’ development, the value of NPQ decreased along with aging, but its increase was observed in the case of infected plants (Fig. 2A, Table 3). It is known from the literature [11, 17] that the treatment of plants with the elicitors of pathogens led to a decrease in the accumulation of a number of photosynthetic proteins and among them is the PsbS subunit of PS II, which is involved in the dispersion of excess light energy via NPQ. A transcription response was observed quite quickly (during the interval of 0-2 hours after the inoculation) [7]. Our experiments demonstrated that even 2 days after the inoculation of fungus spores, a level of NPQ in young infected plants was lower almost twice compared to the control. Assuming that a decrease in the NPQ fluorescence of Chl a is associated with the inhibition of its accumulation in the PsbS subunit, our results may indicate that an inhibitory effect of fungal contamination on the protein synthesis was the highest in young seedlings and it weakened with aging.
Based on the results obtained, it may be assumed that peroxidases are involved in the antioxidant protection of green barley seedlings from the fungal infection at different stages of ontogenesis (Fig. 3). The activity of this enzyme belonging to the oxidase family increased in the case of an infection, while the content of pigments decreased. It should be noted that despite relatively high activity of examined antioxidant enzymes in fungal contamination, the total amount of ROS in the leaf tissue remained at a higher level compared to the control group at all stages of ontogenesis (Table 2). Presumably it could be explained by the fact that in response to the pathogen attack, different ROS species are formed not only in chloroplasts, but also in apoplasts, mitochondria and other cellular compartments. Chloroplast is considered to be the most significant producer of ROS upon initiation and development of cellular death under HR. However, ROS, as byproducts in chloroplasts, are also critical and effective components for plant immunity. ROS not only blocks further colonization by pathogens [44-46], but also act as signaling molecules to reprogram an expression of nuclear defense genes [47]. To weaken the function of chloroplasts, some pathogens directly target host chloroplasts, altering the thylakoid membrane structure and suppressing the production of defense signals, including salicylic acid, NO and ROS.
A high level of LPO in biological membranes is the most obvious symptom of oxidative stress in plants. When ROS levels exceed a plant capacity to scavenge, LPO in biological membranes increases, thereby affecting numerous physiological processes of cell perception [48]. As for the post-pathogen attack, some of the products of lipid peroxidation are reactive electrophiles with a carbonyl group [49]. These electrophiles are a consequence of a ROS impact on membrane lipids or are products arising from lipoxygenase enzyme activity. A view that LPO is solely a destructive process has changed over the past ten years. It was demonstrated that lipid hydroperoxides and other products of lipid degradation, as well as LPO initiators (i.e. ROS), can mediate in the signal transduction cascade [48-52]. Under stress conditions, a typical increase in the ROS content and antioxidant enzymes’ expression take place, including the concentration of lipoxidation products and variability growth exponentially together with reactive carbonyl species (RCS), as well as metabolites resulting from their reaction with proteins (advanced lipoxidation endproducts, ALE). On the contrary, the plants designed as resistant may or may not demonstrate the enhanced levels of ROS and antioxidant enzymes, whereas the levels of lipid oxidation markers as MDA are typically reduced [48]. Thus, the experimental infection of Arabidopsis thaliana leaves with the conidial suspensions of the necrotrophic pathogen Botrytis cinerea [48] resulted in a large decrease in the level of ascorbic acid. The aldehydic product of LPO, 4-hydroxy-2-nonenal, was not observed and in the case of MDA, the levels measured in the infected plants were appreciably lower than in the healthy controls. As stated by these authors, such findings are surprising and demonstrate a difference in the response of A. thaliana to the infection with B. cinerea compared with the tissues of other plant families studied previously. In our experiments, a trend towards a change in the total ROS content and LPO activity in the ontogenesis of green seedlings was opposite, especially for young plants (Tables 2 and 3). This unusual phenomenon is possibly associated not only with a short lifetime of the ROS studied and the activation of antioxidant defense enzymes under pathogenesis (Fig. 3), but also with other metabolic reactions, as shown for Arabidopsis [53].
The protective role phenolic compounds play in the cases where plants are attacked by fungal pathogens is extensively documented [54]. However, in general, performed antifungal phenolics are commonly sequestered in the conjugated form, usually with glycosidic attachments, in vacuoles or organelles in healthy plants. These compounds may also be considered as preformed antibiotics, since the plant enzymes that activate them are already present, but they are separated from their substrates or exist in inactivation forms. In such cases, free phenolics are likely to be much more toxic to the invading organism than the bound forms. Biotrophs may avoid the release of preformed antibiotics, such as phenolics, by minimizing damage to the host, whereas necrotrophs are likely to cause the substantial release of these compounds [54, 55]. Thus, a barley mutant (ant 18-159) was found extraordinary resistant to the Fusarium attack. Indeed, hyphae were unable to penetrate the testa of this mutant due to the presence of antifungal phenolic compounds. We found that the highest content of phenols is typical for 12-day-old barley seedlings characterized by a low content of photosynthetic pigments and low PS II activity (Table 4). Based on the data obtained, it may be assumed that in cases where the photosynthetic activity of chloroplasts is high, a need for polyphenols in plant tissue is reduced.
With inevitable biological scattering and oscillation of almost all of the studied issues in different series of experiments, first of all, it is necessary to consider a direction of correlational interactions between the assessed parameters in order to specify the most informative trends, discuss the relationship of plant immunity and the state and function of photosynthetic apparatus. First, this is a high ratio of a positive correlation (r = + 0.83) between a change in the Chl content and the LPO activity. This suggests that, regardless of the reason that causes a change in the content of pigments, their accumulation occurs simultaneously with the LPO level, at least for a particular variety. It should be noted that during the destruction of pigments in a suspension of chloroplasts in vitro, the stoichiometry of changes in the amount of TBA-active products per Chl unit was inverse ― it increased with a decrease in the Chl a content [56].
A fairly high positive correlation observed between a change in the value of NPQ and a level of LPO activity (r = + 0.71) is rather interesting, not entirely clear though. Our calculations indicate the absence of simple and seemingly logical cause-and-effect relationships and this necessitates further research.
CONCLUSION
The results obtained suggest that in the first leaves of barley, which differ by the state of chloroplasts at different stages of development, a response to fungal infection differs, primarily by the PS II activity, the total content of ROS and polyphenols, and the LPO activity.
It may be assumed that peroxidases are involved in the antioxidant protection of green barley seedlings in cases of fungal infection at different stages of ontogenesis. Moreover, there is a great difference in peroxidase (POX) and ascorbate peroxidase (APX) activation under the fungal attack: an increase in POX activity at the anabolic stage of chloroplast biogenesis and its decrease at the catabolic stage against the background of a constant increase in APX activity.
The highest content of phenols is typical for 12-day-old barley seedlings characterized by a low content of photosynthetic pigments and low PS II activity, which allows us to conclude that high photosynthetic activity of chloroplasts leads to the reduced phenol accumulation in plant tissue under helminthosporiosis. A positive correlation between a change in the Chl and MDA content, as well as between the NPQ level and the MDA content, demonstrated a close relationship between photosynthetic and oxidative parameters in healthy and infected barley seedlings.
Despite the complexity of the interpretation of the results obtained, the state of chloroplasts may be a useful tool to control plant immunity in view of breeding and the development of novel technologies for plant protection.
LIST OF ABBREVIATIONS
APX | = Ascorbate Peroxidase |
B.S. | = Bipolaris Sorokiniana |
DCF | = 2´, 7´-Dichlorfluorescein |
DCFDA | = Dichlorfluorescin Diacetate |
Chl | = Chlorophyll |
HR | = Hypersensitivity Reactions |
HPLC | = High-Performance Liquid Chromatography |
LPO | = Lipid Peroxidation |
MAMP | = Microbe-associated molecular |
MAPKs | = Mitogen-activated protein kinases |
MDA | = Malondialdehyde |
NPQ | = Non-photochemical quenching |
PAM fluorometry | = Pulse Amplitude Modulated fluorometry |
PAMP | = Pathogen-Associated Molecular Patterns |
POX | = Peroxidase |
PS II | = Photosystem II |
qP | = Сoefficient of photochemical quenching of absorbed energy |
ROS | = Reactive Oxygen Species |
RCS | = Reactive Carbonyl Species |
SE | = Standard Error |
ETHICS APPROVAL AND CONSENT TO PARTICIPATE
Not applicable.
HUMAN AND ANIMAL RIGHTS
Not applicable.
CONSENT FOR PUBLICATION
Not applicable.
AVAILABILITY OF DATA AND MATERIALS
Not applicable.
FUNDING
The work was financially supported by the State Program of Scientific Research “Biothecnology” for 2016-2020 (Grant number 1.44).
CONFLICT OF INTEREST
The authors declare no conflict of interest, financial or otherwise.
ACKNOWLEDGEMENTS
The authors thank Shashko Ju. − Head of the Immunity Laboratory, the Republican Unitary Enterprise “Research and Practical Centre for Arable Farming of the National Academy of Sciences of Belarus” for the fungus culture Bipolaris sorokiniana and consultation with respect to its cultivation and the procedure of plants inoculation.